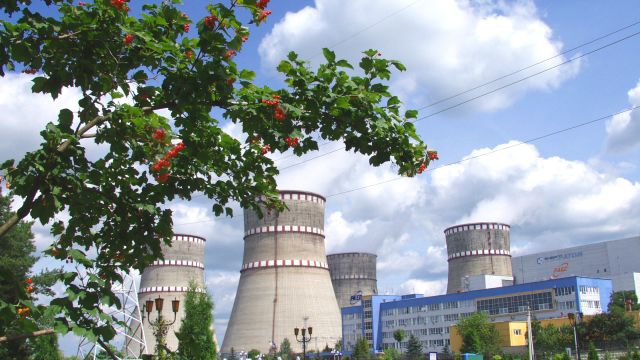
GRS research projects on nuclear safety in Ukraine: Simulations and scenarios of severe VVER reactor accidents
Knowledge of Ukrainian plants essential for proper situation assessments
With the beginning of the Russian invasion of Ukraine, NPPs became the scene of military conflicts for the first time. Since the occupation of the Ukrainian Zaporizhzhya NPP, there have been numerous losses of off-site power (e.g. due to damaged power lines or switching stations), during which the Zaporizhzhya NPP lost its connection to the national grid and had to rely in part on the plant's own emergency diesel generators. In-depth knowledge of the Ukrainian plants is essential in such special situations if the situation on site is to be properly assessed.
In addition to basic knowledge of the structure, functioning and equipment of the Soviet-Russian VVER reactors, this also includes more specific knowledge. This can be derived i.a. from current issues in reactor safety research. Here, GRS is involved in several projects dealing with both the VVER-1000 and the VVER-440 type.
For example, GRS experts are carrying out analyses of hydrogen behaviour in postulated accident scenarios in VVER-440/213 plants and are also involved in modelling the VVER-1000/320 containment. In both cases, GRS is working closely with its Ukrainian partner organisation SSTC NRS; in the latter case, the Bulgarian expert organisation ENPRO Consult is also involved. Both projects, along with other VVER-related research projects, were funded by the German Federal Ministry for the Environment as part of the INT KoNuS programme.
VVER-440: Hydrogen behaviour in postulated accidents
Since the reactor accident at Fukushima Daiichi in March 2011 if not before, the issue of hydrogen has been one of the focal points of reactor safety research and also plays an important role in the European stress test for nuclear power plants. Large quantities of hydrogen, which are produced at high temperatures inside the reactor by the reaction of the zirconium in the cladding tubes of the fuel elements with steam, can lead to flammable gas mixtures and are a key factor when it comes to scenarios involving a possible failure of the so-called accident localisation system. In NPPs with VVER 440/213, the accident localisation system represents the final barrier before radioactivity escapes into the environment in the event of incidents and accidents involving a loss of coolant.
In order to prevent the formation of flammable hydrogen mixtures, all European NPPs were gradually equipped with so-called hydrogen recombiners - if they did not already have them - following the European stress test. These catalytic recombiners are used to oxidise hydrogen with atmospheric oxygen to form steam. Typically, the catalytic converter required for this consists of palladium or platinum, which is vapour-deposited onto a carrier material, such as small beads of aluminium oxide or plates of stainless steel.
Recombiners only help where hydrogen actually accumulates
However, these recombiners only help where hydrogen actually accumulates in the reactor building. In order to find out exactly where these rooms are located, GRS scientists and their Ukrainian partners first created a data set for the specially developed COCOSYS simulation code for Units 1 and 2 of the Rivne NPP.
Figure 1 shows the building cross-section of a VVER-440/213, in which the rooms of the accident localisation system are highlighted in yellow. The current COCOSYS data set models both the accident localisation system and the current status of the plant.
The information required for this was provided by our Ukrainian colleagues. This data set could then be used in accident analyses; in this context, the GRS experts considered various concepts for how passive hydrogen recombiners can be arranged in the accident localisation system.
Formation of flammable gas mixtures can be prevented by retrofitting recombiners
Figure 2 shows the allocation of plant rooms to COCOSYS model zones and their connections with each other. It also shows the allocation of the various recombiner types to the plant rooms for a concept with 63 recombiners. Among other things, COCOSYS analyses were carried out for the accident scenario "Leak in a reactor coolant line with simultaneous total loss of power". With the help of these analyses, the scientists evaluated, among other things
- the calculated hydrogen concentration in the accident localisation system,
- whether and how the flammable gas mixtures form, and
- the effectiveness of installed recombiners.
The results of the accident analyses show that the risk of flammable gas mixtures forming can be effectively contained by retrofitting recombiners. Figure 2 shows that in the concept with 63 recombiners, the calculated gas mixture composition only fulfils the flammability criteria (see flame symbol in the illustration) in the SGBOX1A break room and combustion occurs, although in contrast to the original concept with only 8 recombiners (marked in green), it does not spread to neighbouring rooms.
However, the installation of a total of 15 recombiners in so-called dead-end zones (shaded grey in Figure 2) is ineffective, which can be substantiated by analysing further scenarios.
WWER-1000: Accident scenario with severe core damage and spreading of melt
In a different project, an accident scenario with severe core damage and the spreading of melt was analysed for a VVER-1000/320. One focus of the study was the extent to which the accident sequence is influenced by the level of detail of the spatial modelling of the reactor cavity - in which the reactor pressure vessel (RPV) is located - and the neighbouring rooms. The GRS simulation code COCOSYS was also used for these analyses.
To this end, the scientists first developed a model for the VVER-1000 containment, allowing them to map the physical and chemical phenomena during an accident analysis appropriately and with sufficient accuracy. Figure 3 shows the COCOSYS model consisting of 97 zones.
Scenario: Loss-of-coolant accident
For the analysis, the experts assumed the scenario of a loss-of-coolant accident with a total loss of power (so-called "station blackout"). As a result of the lack of core cooling and the other assumed failures, the reactor core melts after a short time and the RPV fails after 3.25 hours.
After the radial breakthrough of the concrete in the reactor cavity, the core melt moves underneath the steel door into the adjacent room, where Melt Pool 2 (Zone 2) forms. The melt then spreads further on the floor and enters the so-called annulus of the containment, in which Melt Pool 3 (Zone 3) is formed. Immediately after exiting, the melt comes into contact with the water in Zone 3, which leads to high steam production in the containment.
Spreading of the melt
In the simulation, which consists of several variant calculations, the melt spreads over an area of more than 230 square metres and reaches a height of up to 10 centimetres in Zone 2.
The height difference between Zones 2 and 3 calculated from the step and melt pool height significantly influences the water-melt interaction in the annulus, the change between wet and dry states on the melt surface, and the pressure conditions in the containment.
The analysis showed that filtered venting is activated after around 10.6 hours in a scenario with a constant floor height. When modelling the soil geometry more precisely, which includes a 20 cm high step, activation is delayed by 3.3 hours until approx. 13.9 hours. This is due to the contact between the melt and the water, which is influenced by the height difference between Zones 2 and 3. This time shift is relevant for the calculated source term as it determines the quantity and type of radioactive nuclides released. The additional time until venting allows radioactive aerosols to deposit and decay, which in turn influences the potential radioactive contamination of the environment.
International cooperation to be continued
The results of the investigations outlined here show that the inclusion and consideration of plant details in the modelling have a significant effect on the accident sequences both in the accident localisation systems of NPPs with VVER-440 and in the containments of VVER-1000 plants. The successful international cooperation to date in the field of accident analyses should therefore be continued in the future in order to minimise further uncertainties in the calculation codes and in plant modelling for the performance of accident analyses, to further improve plant understanding and to keep knowledge of the condition of NPPs with VVER up to date.