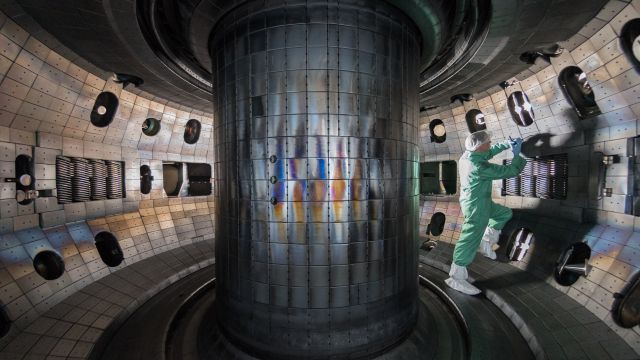
Fusion - what is behind it?
Scientists around the world are working on ways to realise this vision. But the list of technical challenges before fusion can be utilised on an industrial scale is long. So far, only large-scale research facilities are being operated, which are still a long way from the goal of net energy production - they still consume more energy during operation than is generated by fusion.
What is behind it? The basic principle of fusion
In contrast to nuclear fission, fusion uses energy to cause light atomic nuclei (e.g. hydrogen) to fuse together to form heavier atomic nuclei (e.g. helium). A large amount of energy is released during the fusion process. For example, stars such as the sun obtain their energy from nuclear fusion. When deuterium reacts with tritium (both hydrogen isotopes), for instance, an alpha particle (helium nucleus) and a neutron with high kinetic energy are released. The deuterium-tritium reaction is of particular interest as the conditions for this reaction are the most technologically feasible in a technical facility. It is therefore the favoured option for the fusion process.
From fusion energy to electricity from the socket
The neutron released during the fusion process accounts for most of the energy generated (around 80 per cent). This energy is largely converted into heat in the so-called blanket (inner lining of the vacuum chamber), which is then converted into electrical energy using a conventional water-steam cycle with an attached generator. According to the Max Planck Institute for Plasma Physics, one gram of fuel could generate around 90,000 kilowatt hours (kWh) of energy. This corresponds to the combustion heat of 11 tonnes of hard coal.
Conditions for fusion
The so-called Coulomb force ensures that two positively charged atomic nuclei repel each other. This represents a barrier to the fusion process that must be overcome. In fusion power plants, pressure and temperature are used to create conditions that bring the nuclei so closely together despite this repulsion that the probability of fusion increases. For example, hydrogen in the centre of the sun fuses into helium at a pressure of 200 billion bar and 15 million Kelvin. One measure of this probability is the so-called reaction cross-section. This depends on the energy of the particles that collide. As soon as this repulsion is overcome, the strong interaction between the nuclei ensures that the nuclei merge, i.e. fuse.
Plasma
The state in which matter finds itself under the conditions necessary for fusion is called "plasma". It is also known as the fourth state of matter. Here, a certain proportion of the atoms (in the fuel mixture under consideration here, these are hydrogen isotopes) are ionised, i.e. positively charged, whereby some electrons (in the case of hydrogen isotopes, it is only one) are no longer bound to the atomic shell.
Lawson criterion
The so-called Lawson criterion is a decisive benchmark for a self-sustaining fusion reaction, particularly with regard to industrial utilisation. Whether the Lawson criterion is met depends on the temperature, density and confinement time of the plasma. The confinement time is the specific time after which the heat in the plasma is lost. Only then can the fusion reaction sustain itself.
In principle, the Lawson criterion can be fulfilled in two ways: on the one hand by the longest possible confinement time at a relatively low density, and on the other hand by the highest possible density and a rather short confinement time.
Magnetic confinement fusion and inertial confinement fusion - the two major concepts
The first approach is achieved by confining the plasma in a magnetic field. Here, the density can assume comparatively low values, but the confinement time can be extended, albeit with considerable effort. This involves so-called magnetically confined fusion plasmas, which operate under the concept of magnetic fusion energy (MFE).
The second idea is to heat a fuel capsule with a high fuel density but short confinement time to fusion conditions. Most concepts for this so-called inertial confinement are based on bombarding a fuel capsule with laser beams, whereby the outermost layers of the capsule vaporise very quickly. The resulting reaction force causes the fuel pellet to implode, so that fusion conditions are achieved in the centre. This approach is known as inertial fusion energy (IFE) or inertial confinement fusion (ICF).
In fusion research, MFE is generally considered to be much more advanced, although current progress in IFE has also recently made the headlines.
Magnetically confined fusion plasmas - magnetic fusion
MFE involves confining an ionised gas in a magnetic field cage. However, charged particles can only be trapped perpendicular to the magnetic field lines; they can continue to move freely along them. For complete confinement, in the most common concepts of magnetic fusion (see Figure 1), both ends of a configuration are brought together so that a ring-shaped configuration is created, similar to the shape of a donut.
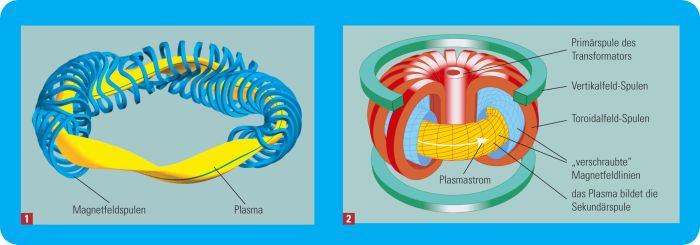
The particles move within this circular apparatus and are largely kept away from the walls due to the magnetic field lines. Contact of the plasma with the container walls would lead to immediate cooling of the plasma and thus to the end of the fusion process.
The confinement of the ionised gas in a magnetic field cage is possible because the velocity component of charged particles perpendicular to the magnetic field is subject to the so-called Lorentz force, so that a deflection occurs there. Ultimately, the particles move around the magnetic field lines in spiral paths (technically: they gyrate). To put it simply, they can move parallel to the magnetic field, but not perpendicular to it. In order to confine them, the particles at the end of an apparatus must be reflected with the help of so-called mirror machines or both ends of an assembly must be brought together again so that a torus-shaped assembly is created - comparable to a life ring or donut. In this way, the particles would still move along the magnetic field lines, but they would do so within the circular apparatus and be kept away from the cold walls. Contact between the plasma and the cnfinement walls would lead to immediate cooling of the plasma and thus to a collapse of the fusion process.
On closer inspection, however, particles actually also perform a movement perpendicular to the magnetic field lines, which is called drift. There are different types of drift, which are usually named after their cause. For example, there is an ExB drift, which is due to the existence of electric fields (E), or a diamagnetic drift, which is caused by a pressure gradient (Ñp). This is precisely what leads to charge separation, which generates an electric field, which in turn leads to an ExB drift of the particles, so that the plasma threatens to disappear from the confinement area. To compensate for this phenomenon, the magnetic field lines in a torus-like plasma chamber are twisted helically along the chamber so that the charge separation can dissipate via so-called Pfirsch-Schlüter currents.
There are two main approaches to the technical realisation of the MFE: the tokamak designed by I. E. Tamm and A. D. Sakharov and the stellarator devised by Lyman Spizer in the USA.
Tokamak
The first tokamak (Russian acronym for toroidal chamber in magnetic coils) was realised in the former Soviet Union. The characteristic feature of a tokamak is its ring-shaped vacuum chamber (see Figure 1), which is surrounded by circular (toroidal) magnetic field coils.
The torsion of the magnetic field lines in the tokamak is achieved by driving a plasma current in the chamber, whose own magnetic field (poloidal) is superimposed on the magnetic field of the coils (toroidal). The plasma current is in turn driven by the central transformer, which induces a voltage via a time-varying magnetic field strength. This time-varying magnetic field is generated by a current in the transformer, the strength of which is limited. Once the maximum current strength has been reached, the transformer must be switched off and start the build-up of current supply again. Due to this fact, a tokamak does not generate a continuous current, but rather a "pulsed" current for several minutes at a time. Research is therefore currently focussing in particular on the question of how these pulse sequences can be extended or even how continuous operation can be implemented in order to achieve continuous power generation.
ITER
A well-known example of a tokamak concept is the fusion project ITER (originally for: International Thermonuclear Experimental Reactor), in which 35 countries have joined forces for research purposes. The experimental fusion plant of the same name has been under construction at Cadarache in southern France since 2007. The first fusion plasma is planned in 2035.
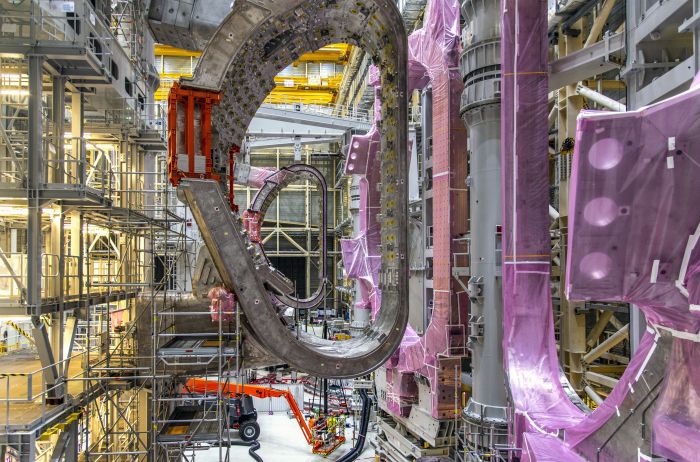
Parallel to ITER, intensive work is already underway on concepts for a follow-up demonstration facility (DEMO for short). The aim is to demonstrate both the economic viability of the plant and the function of the closed fuel cycle (in the form of incubation of the fuel component tritium). DEMO plants are usually publicly owned until commercial utilisation is "proven".
JET
JET (Joint European Torus) was the largest tokamak experiment in the world and is located in Culham in the United Kingdom. Under the management of EUROfusion, JET was shared by more than 31 European research organisations. JET was one of the few facilities capable of accommodating the favoured fuel pairing of deuterium-tritium so that fusion plasmas could be produced. On 9 November 1991, a fusion plasma with an output of 1.7 megawatts (MW) was briefly achieved for the first time. In several test campaigns, the output was increased to 16 MW. After 40 years and several records in terms of fusion power and pulse duration, the facility was decommissioned at the end of 2023.
ASDEX -Upgrade
In Germany, the large-scale research facility ASDEX-Upgrade is operated by the Max Planck Institute for Plasma Physics. It is an upgraded version of the ASDEX tokamak (axially symmetrical divertor experiment), which went into operation in 1991. ASDEX-Upgrade will be used to investigate fundamental questions of fusion under power plant-like conditions (e.g. with regard to plasma properties, loads on the walls) and in this way develop the physical foundations for ITER and DEMO.
Further tokamak concepts
JT-60SA (J: Japan, T: Tokamak) is also a tokamak research facility. It is operated by a consortium of Japanese and European organisations in Naka, Japan. Until the completion of ITER, the JT-60SA is the world's largest tokamak, with which plasma could be generated for the first time in October 2023. In contrast to ITER, the JT-60SA uses light hydrogen and deuterium instead of a tritium-deuterium mixture. Research here is focused on plasma physics. Another tokamak experiment is being conducted with KSTAR (Korea Superconducting Tokamak Advanced Research) in South Korea.
The Massachusetts Institute of Technology (MIT) in the US is developing the SPARC (soonest/smallest privately-funded, affordable, robust, compact) fusion demonstration facility together with the start-up Commonwealth Fusion Systems (CFS). It is based on high-temperature superconductor magnets (HTC). These allow a higher limiting current and therefore a higher magnetic field, which should lead to future facilities being smaller and cheaper to build.
The so-called spherical tokamak is another variant of the tokamak. It has almost spherical symmetry and is smaller overall than other tokamak facilities with a similar output. This type includes the experimental MAST (mega ampere spherical tokamak) and MAST-Upgrade facilities in the UK. A demonstration power plant is currently being designed for the spherical tokamak (STEP - Spherical Tokamak for Energy Production), which is due to be connected to the grid in the 2040s.
In the summer of 2023, China announced that the HL-3 research reactor (with so-called high-confinement mode, or H-mode for short) was operating for the first time with a plasma current of 1 million amperes - a record for China's nuclear fusion facilities with magnetic confinement. Compared to its "predecessor" L-mode (low-confinement mode), H-mode ensures better confinement of the plasma and is therefore more favourable in terms of energy. It will also be the standard operating mode for ITER, which is currently under construction.
Stellarator
Stellarators differ from tokamaks mainly in the configuration and shape of the magnetic coils (see Figure 1), which ensure the torsion of the magnetic field lines. In the Wendelstein 7-X project at Greifswald - the world's largest stellarator-type fusion facility - the magnetic field is generated by 50 specially shaped, superconducting magnetic coils. "In a stellarator, the helical torsion of the magnetic field lines is generated exclusively by external coils. A stellarator therefore manages without a longitudinal current in the plasma and therefore without a transformer," is how the Max Planck Institute for Plasma Physics (IPP) describes the special features on its website. In contrast to the tokamak, this should allow the continuous operation of a fusion facility.
Proxima Fusion, a spin-off of the IPP, is planning to build fusion power plants based on quasi-isodynamic (QI) stellarators with high-temperature superconductors. The "ground-breaking" results from the Wendelstein 7-X facility will be the starting point.
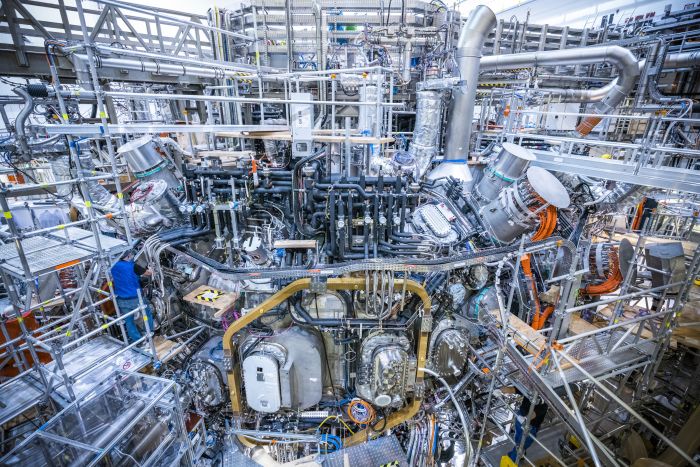
Inertial confinement/laser fusion
In contrast to the magnetic configurations used in the tokamak and stellarator, in laser fusion strong laser pulses act on a small capsule filled with fusion fuel (e.g. deuterium and tritium). This causes the surface to vaporise and detach, creating a reaction force that compresses the pellet to such an extent that the conditions for an ignition of fusion are achieved inside it. The resulting "hot spot" spreads into the still unburnt areas of the fuel capsule, so that further burn-up can take place without any additional energy supply from outside.
A distinction is made between direct and indirect propulsion. The former works by firing laser pulses directly at the fuel capsule. In indirect propulsion, on the other hand, the fuel capsule is located in a cavity. The laser beams first hit the inside of this cavity and generate X-rays there, which then irradiate the fuel capsule homogeneously from all sides. In this so-called radiation bath, the surface of the fuel capsule is also vaporised (ablation). This indirect process is associated with relatively high losses. A power plant could be operated economically if this implosion process were to take place at a frequency of 10 Hertz (equivalent to 10 implosions per second). Such a frequency would mean quasi-continuous operation. However, existing plants are purely "single shot plants", in which the fuel capsule is located on a sample holder, whereas the capsules in a power plant would have to be shot into the reaction chamber at the frequency mentioned.
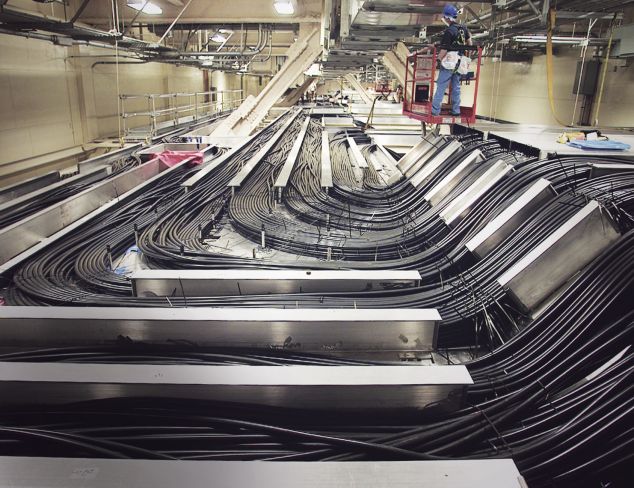
One example of such a single-shot facility is the National Ignition Facility (NIF) at the Lawrence Livermore National Laboratory in the USA (see Figure 4). The facility is mainly used for nuclear weapons research.
At the end of 2022, the Lawrence Livermore National Laboratory announced that, for the first time, they had been able to generate more energy (3 megajoules, MJ) in one of their experiments on inertial confinement fusion than they had to supply to start the reaction using laser pulses (2 MJ) (see: Lawrence Livermore National Laboratory). However, in relation to the total energy required to operate the system, this amount of fusion energy generated is still only a fraction (around one per cent).
The Laser Mégajoule (LMJ) facility in France is also operated for the purpose of nuclear weapons research. However, aspects relevant to a fusion power plant are also to be investigated there, for example the demonstration of direct and indirect propulsion of fusion reactions.
Challenges of Fusion
The development of fusion facilities on a large industrial scale is driven on the one hand by the desire for greater independence from conventional energy sources - in particular coal, gas and nuclear energy from nuclear fission - and on the other hand by the virtually inexhaustible energy potential of this technology, which can help to meet the world's growing demand for energy. Nevertheless, research into fusion to date shows that there is still a long way to go before the technology can be utilised commercially. The challenges here, however, lie primarily in technical aspects, for example with regard to the ageing of the material. The physical understanding of the process, on the other hand, is considered to be sufficiently researched for the technology
The challenges include, for example, controlling the numerous plasma instabilities - in simple terms, disturbances - which initially deform the magnetic cage and can lead to a breakdown of the plasma discharge and thus the generation of energy. A further challenge is to achieve a fusion power plant that operates as continuously as possible.
Tritium, which is a fuel component, requires special precautions during handling and storage. In addition, efficient methods for producing tritium have yet to be verified: tritium must be continuously re-bred in a fusion plant (known as a closed fuel cycle), whereas deuterium can be extracted from water.
Another aspect is the neutron radiation emitted during the nuclear fusion process (see also the first section on electricity generation). The neutrons released during the fusion process are captured in the blanket by atomic nuclei of the structural materials. On the one hand, this is desirable, as the fuel component tritium is to be bred via the neutron capture, but the capture of structural materials also leads to ageing and activation of these materials (see Activation). The materials used must therefore be designed in such a way that they can withstand the neutron radiation and at best shield it.
The ageing of the materials and the consumption of lithium mean that the blanket has to be replaced several times during the power plant's lifetime. Further research is needed to control or minimise the ageing of the materials. This applies to both laser and magnetic fusion. In Granada (Spain), the construction of an irradiation facility is planned in which materials will be analysed for precisely these properties (see also: International Fusion Materials Irradiation Facility - Demo Oriented Neutron Source (Ifmif Dones)).
And last but not least, the economic viability (construction, maintenance, electricity price, etc.) also plays a role in all considerations relating to fusion energy. One of the decisive factors is the extent to which the results from the currently planned experimental fusion plants can be applied to larger, commercial plants. This is the area in which the DEMO concepts operate.
Despite these challenges, progress in fusion research is promising and the international research community is continuing to work intensively on overcoming these technical rather than fundamental hurdles.
Are fusion power plants safer than nuclear power plants?
The hazard potential posed by fusion plants is considered to be considerably lower than that of reactors that use nuclear fission to generate energy. A major difference lies in the type and quantity of radioactive inventory that could be released in the event of an accident.
During nuclear fission, heavy atomic nuclei such as uranium-235 are split. What remains are lighter nuclei, so-called fission products. In addition, actinides (e.g. plutonium) are produced through breeding reactions. Most of these are highly radioactive and generate a high decay power even after the chain reaction has ended, which must be removed. If this does not succeed, e.g. because there is insufficient cooling available or other emergency measures are not effective, a reactor core may melt. Depending on the course of the accident, radionuclides may then be released into the environment.
No fission products or actinides are produced during fusion. The result of the fusion of two hydrogen nuclei is a non-radioactive helium nucleus. It is only power plant components that are activated. In addition to the activated components, the activity inventory of a fusion plant consists of dust produced by erosion of the walls facing the plasma (e.g. blanket wall) and activated corrosion products in the coolant. In addition, the radioactive fusion fuel tritium contributes to the total inventory.
Nuclear fission produces transuranic elements such as plutonium, which has a half-life of 24,000 years. Nuclear fusion does not produce such long-lived waste. Accordingly, a significantly shorter period of time is assumed for which safe storage of this waste will be required. However, the quantities of waste produced are considerably larger than those resulting from nuclear fission. It is therefore planned to recycle this material once the radiation has sufficiently decayed.
Another advantage of nuclear fusion over nuclear fission is that no uncontrolled chain reaction can occur during nuclear fusion. In order to maintain nuclear fusion, the stability of the plasma must be guaranteed, for example by maintaining the magnetic field. This requires an immense amount of effort. If there are disturbances or deviations and the plasma comes into contact with the wall of the blanket, the fusion would immediately stop on its own. A "runaway" of the reactor, as e.g. in the reactivity accident at Chernobyl, is therefore impossible for physical reasons.
GRS has already dealt with various aspects of fusion energy. For example, the regulatory framework for fusion plants was analysed in a project commissioned by the European Commission (EU) together with the Karlsruhe Institute of Technology (KIT). In co-operation with the KIT, several incident and accident simulations have also been carried out with the MELCOR integral code used in nuclear technology.
Another research research project of the Federal Ministry for the Environment, Nature Conservation, Nuclear Safety and Consumer Protection (BMUV) focused on safety-related issues. The focus here was on the systems and measures envisaged in DEMO concepts to guarantee the basic and selected supporting safety functions.